Study finds topological materials
could boost the efficiency of thermoelectric devices.
Jennifer Chu | MIT
News Office
COMMENT
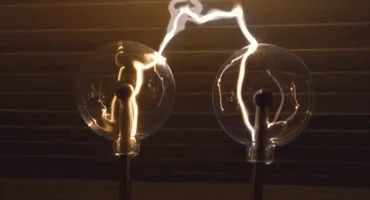
Thermoelectric devices
are made from materials that can convert a temperature difference into
electricity, without requiring any moving parts — a quality that makes
thermoelectrics a potentially appealing source of electricity.
The phenomenon is reversible: If electricity is applied to a thermoelectric device, it can produce a temperature difference. Today, thermoelectric devices are used for relatively low-power applications, such as powering small sensors along oil pipelines, backing up batteries on space probes, and cooling minifridges.
The phenomenon is reversible: If electricity is applied to a thermoelectric device, it can produce a temperature difference. Today, thermoelectric devices are used for relatively low-power applications, such as powering small sensors along oil pipelines, backing up batteries on space probes, and cooling minifridges.
But scientists are
hoping to design more powerful thermoelectric devices that will harvest heat —
produced as a byproduct of industrial processes and combustion engines — and
turn that otherwise wasted heat into electricity. However, the efficiency of
thermoelectric devices, or the amount of energy they are able to produce, is
currently limited.
Now researchers at MIT
have discovered a way to increase that efficiency threefold, using
“topological” materials, which have unique electronic properties.
While past work has suggested that topological materials may serve as efficient thermoelectric systems, there has been little understanding as to how electrons in such topological materials would travel in response to temperature differences in order to produce a thermoelectric effect.
While past work has suggested that topological materials may serve as efficient thermoelectric systems, there has been little understanding as to how electrons in such topological materials would travel in response to temperature differences in order to produce a thermoelectric effect.
In a paper published
this week in the Proceedings of the National Academy of Sciences,
the MIT researchers identify the underlying property that makes certain
topological materials a potentially more efficient thermoelectric material,
compared to existing devices.
“We’ve found we can
push the boundaries of this nanostructured material in a way that makes
topological materials a good thermoelectric material, more so than conventional
semiconductors like silicon,” says Te-Huan Liu, a postdoc in MIT’s Department
of Mechanical Engineering.
“In the end, this could be a clean-energy way to help us use a heat source to generate electricity, which will lessen our release of carbon dioxide.”
“In the end, this could be a clean-energy way to help us use a heat source to generate electricity, which will lessen our release of carbon dioxide.”
Liu is first author of
the PNAS paper, which includes graduate students Jiawei Zhou,
Zhiwei Ding, and Qichen Song; Mingda Li, assistant professor in the Department
of Nuclear Science and Engineering; former graduate student Bolin Liao, now an
assistant professor at the University of California at Santa Barbara; Liang Fu,
the Biedenharn Associate Professor of Physics; and Gang Chen, the Soderberg
Professor and head of the Department of Mechanical Engineering.
A path freely traveled
When a thermoelectric
material is exposed to a temperature gradient — for example, one end is heated,
while the other is cooled — electrons in that material start to flow from the
hot end to the cold end, generating an electric current.
The larger the temperature difference, the more electric current is produced, and the more power is generated. The amount of energy that can be generated depends on the particular transport properties of the electrons in a given material.
The larger the temperature difference, the more electric current is produced, and the more power is generated. The amount of energy that can be generated depends on the particular transport properties of the electrons in a given material.
Scientists have
observed that some topological materials can be made into efficient
thermoelectric devices through nanostructuring, a technique scientists use to
synthesize a material by patterning its features at the scale of nanometers.
Scientists have thought that topological materials’ thermoelectric advantage comes from a reduced thermal conductivity in their nanostructures. But it is unclear how this enhancement in efficiency connects with the material’s inherent, topological properties.
Scientists have thought that topological materials’ thermoelectric advantage comes from a reduced thermal conductivity in their nanostructures. But it is unclear how this enhancement in efficiency connects with the material’s inherent, topological properties.
To try and answer this
question, Liu and his colleagues studied the thermoelectric performance of tin
telluride, a topological material that is known to be a good thermoelectric
material. The electrons in tin telluride also exhibit peculiar properties that
mimick a class of topological materials known as Dirac materials.
The team aimed to
understand the effect of nanostructuring on tin telluride’s thermoelectric
performance, by simulating the way electrons travel through the material. To
characterize electron transport, scientists often use a measurement called the
“mean free path,” or the average distance an electron with a given energy would
freely travel within a material before being scattered by various objects or
defects in that material.
Nanostructured
materials resemble a patchwork of tiny crystals, each with borders, known as
grain boundaries, that separate one crystal from another. When electrons
encounter these boundaries, they tend to scatter in various ways. Electrons
with long mean free paths will scatter strongly, like bullets ricocheting off a
wall, while electrons with shorter mean free paths are much less affected.
In their simulations,
the researchers found that tin telluride’s electron characteristics have a
significant impact on their mean free paths. They plotted tin telluride’s range
of electron energies against the associated mean free paths, and found the
resulting graph looked very different than those for most conventional
semiconductors.
Specifically, for tin telluride and possibly other topological materials, the results suggest that electrons with higher energy have a shorter mean free path, while lower-energy electrons usually possess a longer mean free path.
Specifically, for tin telluride and possibly other topological materials, the results suggest that electrons with higher energy have a shorter mean free path, while lower-energy electrons usually possess a longer mean free path.
The team then looked
at how these electron properties affect tin telluride’s thermoelectric
performance, by essentially summing up the thermoelectric contributions from
electrons with different energies and mean free paths. It turns out that the
material’s ability to conduct electricity, or generate a flow of electrons,
under a temperature gradient, is largely dependent on the electron energy.
Specifically, they
found that lower-energy electrons tend to have a negative impact on the
generation of a voltage difference, and therefore electric current. These
low-energy electrons also have longer mean free paths, meaning they can be
scattered by grain boundaries more intensively than higher-energy electrons.
Sizing down
Going one step further
in their simulations, the team played with the size of tin telluride’s
individual grains to see whether this had any effect on the flow of electrons
under a temperature gradient. They found that when they decreased the diameter
of an average grain to about 10 nanometers, bringing its boundaries closer
together, they observed an increased contribution from higher-energy electrons.
That is, with smaller grain sizes, higher-energy electrons contribute much more to the material’s electrical conduction than lower-energy electrons, as they have shorter mean free paths and are less likely to scatter against grain boundaries. This results in a larger voltage difference that can be generated.
What’s more, the
researchers found that decreasing tin telluride’s average grain size to about
10 nanometers produced three times the amount of electricity that the material
would have produced with larger grains.
Liu says that while
the results are based on simulations, researchers can achieve similar
performance by synthesizing tin telluride and other topological materials, and
adjusting their grain size using a nanostructuring technique.
Other researchers have suggested that shrinking a material’s grain size might increase its thermoelectric performance, but Liu says they have mostly assumed that the ideal size would be much larger than 10 nanometers.
Other researchers have suggested that shrinking a material’s grain size might increase its thermoelectric performance, but Liu says they have mostly assumed that the ideal size would be much larger than 10 nanometers.
“In our simulations,
we found we can shrink a topological material’s grain size much more than
previously thought, and based on this concept, we can increase its efficiency,”
Liu says.
Tin telluride is just
one example of many topological materials that have yet to be explored. If
researchers can determine the ideal grain size for each of these materials, Liu
says topological materials may soon be a viable, more efficient alternative to
producing clean energy.
“I think topological
materials are very good for thermoelectric materials, and our results show this
is a very promising material for future applications,” Liu says.
This research was
supported in part by the Solid-State Solar Thermal Energy Conversion Center, an
Energy Frontier Research Center of U.S. Department of Energy; and the Defense
Advanced Research Projects Agency (DARPA).